Featured
Mighty Mitochondria
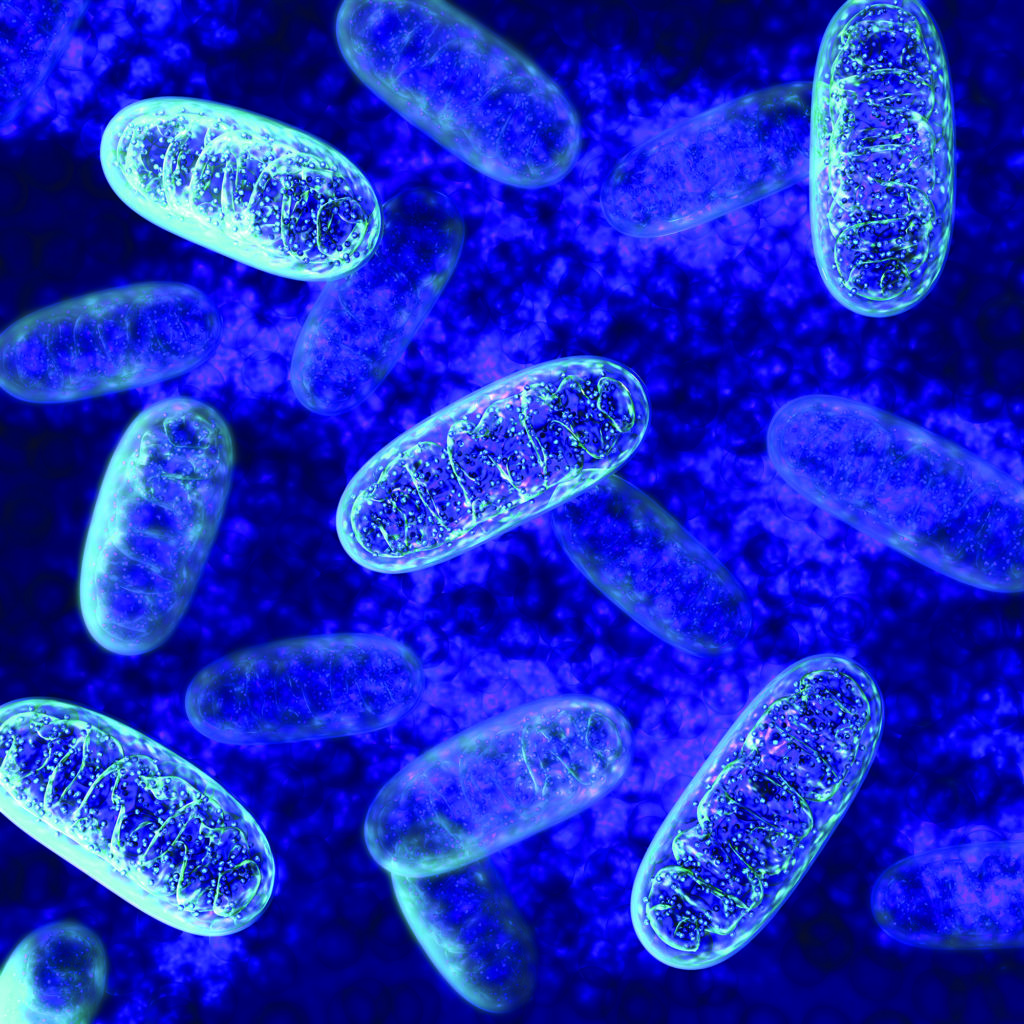
Mitochondria have attracted the fascination of biologists for decades, from their discovery by Swiss histologist, anatomist, and physiologist Albert von Kölliker in the mid-1800s through the pioneering studies of bioenergetics in the 1950s, 1960s, and 1970s. In more recent years, many psychological scientists and neuroscientists have also become fascinated by the role of these specialized cellular structures in brain processes—specifically, changes in the aging brain. Several multidisciplinary research explorations have been reported in APS journals. For example, Bonnie J. Kaplan (University of Calgary) and colleagues investigated the relationship between mitochondrial functioning and mental health in a 2015 article in Clinical Psychological Science, and in a 2019 article in Current Directions in Psychological Science, APS Fellow David Geary (University of Missouri) proposed that the functioning of mitochondria might link intelligence, health, and aging.
Here’s a look at emerging research at the intersection of biology, psychological science, and neuroscience on the organelle that continues to fascinate researchers across fields.
Mitochondria might spend their days as the “powerhouses of the cell” but clearly moonlight in an array of other activities.
Pagliarini and Rutter (2013)
From powerhouses of the cell to agents of cellular death
Mitochondria are cell parts—organelles—with a characteristic double membrane. The number of mitochondria in a cell varies by organism, tissue, and cell type. Mitochondria exist in all human cells except red blood cells.
One of the most striking facts about mitochondria is that they have some DNA of their own (in humans, it is supposedly inherited only from the mother, although a recent study found possible evidence for paternal inheritance of mitochondrial DNA; Luo et al., 2018); they also possess the ability to transcribe DNA and synthesize proteins. These and other unique characteristics make mitochondria similar to bacteria; in fact, researchers have proposed that mitochondria were originally bacteria that ended up establishing a symbiotic relationship with microbes—living inside them, providing them with energy, and ultimately allowing them to evolve into organisms composed of multiple cells with nuclei, including humans (Kramer & Bressan, 2018).
Beginning with the first observation of mitochondria in the 1800s and through the late 1970s, scientists uncovered many of the details on how mitochondria generate energy (adenosine triphosphate, or ATP) and power cells. But by the 1980s, despite that decades-long interest, mitochondria were no longer a hot research topic. In 1998, however, Science published a special issue that revived the scientific community’s enthusiasm. At the time, several labs had established that mitochondria and mitochondrial proteins played an important role in programmed cell death (for a review, see Green & Reed, 1998).
Exploring the Mitochondrion
Mitochondria are cell organelles that exist in most eukaryote organisms (i.e., living organisms composed of one or more cells whose genetic material is contained within a distinct nucleus). The number of mitochondria in a cell varies; they exist in all human cells, except red blood cells, for example, and are especially abundant in liver, muscle, and neuronal cells (e.g., Rango & Bresolin, 2018).
Each mitochondrion is composed of different parts, including an outer membrane, an inner membrane, cristae (formed by the foldings of the inner membrane), and a matrix (the fluid-filled space within the inner membrane). The mitochondrion’s membranes are composed of proteins and polarized layers of lipid molecules (i.e., with a positive charge on one side of the membrane and a negative charge on the other side).
Timeline of mitochondria discovery:
• Around 1857: Albert von Kölliker, a Swiss researcher known mostly for his studies of the neuron and the inner structure of the brain, appears to have been the first to identify groups of granules with membrane (i.e., mitochondria) in the cells of insect muscles (Lehninger, 1964).
• 1890: German histologist Richard Altmann recognized the pervasive presence of these granules, concluding that they were elementary organisms living inside cells and carrying out vital functions. He called them “bioblasts.”
• 1898: Carl Benda, a German microbiologist and pioneer in the use of the microscope to study the internal structure of cells, observed hundreds of small bodies forming long chains in the cytoplasm of eukaryotic cells. He called these structures mitochondria (mitochondrion
in the singular)—the combination of the Greek words “mitos” and “chondros,” meaning thread and granule, respectively.
• 1940–1946: Belgian-American cell biologist Albert Claude used refined centrifugation methods to isolate mitochondria from the rest of the cell.
• 1948–1951: American biochemist Albert Lehninger and students Eugene Kennedy and Morris Friedkin demonstrated the bioenergetic role of mitochondria in the cell, including oxidative phosphorylation, the chemical process in which cells use enzymes to oxidize nutrients and produce energy.
• 1952–1953: Romanian-American biologist George Palade, acclaimed as the founder of modern cell biology, published the first high-resolution images of mitochondria, allowing a detailed description of the organelles.
• 1957: American cell biologist Philip Siekevitz wrote “Powerhouse of the Cell” for Scientific American, coining a term still in use today.
For historical reviews, see Ernster & Schatz (1981) and Pagliarini & Rutter (2013).
In the following decades, researchers went on to detail the role of mitochondria in cell death and aging and how mitochondrial dysfunction is involved in both rare and common human diseases. Beyond their role in bioenergetic processes, or how energy is supplied to and used by cells, mitochondria play a big role in the storage of calcium ions, which are important for signaling between cells, the coordination of hormones, and the release of neurotransmitters. Given their important role in cell metabolism, mitochondrial dysfunctions have been related to diseases affecting virtually every organ and system in our bodies, and in 1994, the term “mitochondrial medicine” came into use (Kaplan et al., 2015). Moreover, given the susceptibility of mitochondrial DNA to oxidative stress, mutation, and the high-energy demands of the brain, it is not surprising that mitochondrial dysfunction in mitochondrial DNA has been associated with some mental disorders (Kaplan et al., 2015), attracting the attention of neuroscientists and psychological scientists. The resulting research has shown that “mitochondria might spend their days as the ‘powerhouses of the cell’ but clearly moonlight in an array of other activities,” wrote David J. Pagliarini (Washington University School of Medicine at St. Louis) and Jared Rutter (University of Utah) in 2013.
Mitochondria in the brain
In a 2018 article in Perspectives on Psychological Science, Peter Kramer and Paola Bressan (University of Padua) reviewed the evidence for associations between mitochondria functioning and mental dysfunction. For instance, they noted, mitochondria provide energy for neurons to fire and help to regulate the chemical reactions required for this process. Every time a neuron fires, sodium ions enter it. If enough sodium enters, channels open to let calcium ions enter the neuron as well. For the neuron to fire again, both sodium and calcium ions must leave it. Mitochondria then temporarily lock the calcium in and provide energy for other cell structures to also lock the calcium ions. But if the mitochondria happen to accumulate too much calcium, they will destroy themselves and the host neuron. This can occur when neuron firing is excessive, as in the case of epileptic seizures.
When this process occurs as it is supposed to, rising calcium levels can make a neuron more responsive to future stimulation. Likewise, reduced calcium levels can make neurons less responsive. Mitochondria affect these responsiveness changes, known as “synaptic plasticity,” by either strengthening or eliminating energy connections. ATP can also function as a neurotransmitter—for example, signaling pain by telling the cells to “spill” ATP after an injury and activating receptors outside of the affected cells (Wirkner et al., 2007). In addition, mitochondria produce precursors of steroid hormones and neurosteroids that can affect learning, memory, and mental health. And when hormones and neurotransmitters are excessive or no longer needed, mitochondria have the task of breaking them down or storing them away.
Consequences of dysfunctional mitochondria
What happens when mitochondria become damaged or dysfunctional? Citing Boesch et al. (2011), Kramer and Bressan noted that not only can damaged mitochondria be repaired, but if the repair fails, parts that are still working can be recycled. Pairs of malfunctioning mitochondria fuse together, reconfigure, and come apart again, creating one mitochondrion with purely functional components and another with components that are damaged or destroyed (Youle & van der Bliek, 2012). However, cells with too many dysfunctional mitochondria become dysfunctional themselves; at that stage, they instruct their mitochondria to kill them and then self-destruct. When this process is disrupted, disease can result.
Because the human brain consumes at least 20% of the energy mitochondria generate and neurons’ oxidative metabolism is 10 times faster than that of other cells (Bélanger et al., 2011), mitochondria dysfunction can affect it greatly. Neurons are always using energy, especially at synapses, and when mitochondria become dysfunctional, they have to be destroyed and replaced, either at the synapse or after being transported away. Failures in those processes of destruction and transport have been linked to neurodegenerative diseases (Martinez-Vicente, 2017), the onset of both Alzheimer’s and Parkinson’s (Correia et al., 2016; Shlevkov & Schwarz, 2017), and even schizophrenia and depression (Deheshi et al., 2013), Kramer and Bressan noted.
Dysfunctional mitochondria can produce higher levels of reactive oxygen species (ROS; reactive chemical molecules that contain oxygen and are generated by normal breathing processes), which cause oxidative stress and can damage cell structures, DNA, RNA, and proteins (Lei et al., 2014). ROS-induced damage contributes to cell death, neurodegenerative diseases, and even normal aging. Higher concentrations of ROS have been linked to attention-deficit/hyperactivity disorder (ADHD; Ceylan et al., 2012), bipolar disorder (Andreazza et al., 2008), paranoid schizophrenia (Dietrich-Muszalska et al., 2012), and autism spectrum disorder (Frye & Rossignol, 2011).
Additionally, a 2008 study connected mitochondrial function with depression and somatic symptoms. Specifically, Gardner and Boles showed that reduced ATP production (as noted, a mitochondrial function) was linked to more severe somatic symptoms in patients with chronic depression. Given these results, “severity of somatic complaints may be a marker of low ATP production and perhaps also the severity of mental symptoms,” wrote Kaplan and colleagues in 2015. Kramer and Bressan also explored how the consequences of dysfunctional mitochondria for neuronal functioning may help to explain why “schizophrenia patients are often depressed, autism patients are often anxious, Down syndrome patients tend to develop premature dementia, and current depression predicts dementia later on.”
When mitochondria age
Understanding how mitochondria contribute to human brain functions may also shed light on their influence in both healthy brain aging and some aging-related neurological diseases.
Biochemist Denham Harman characterized aging as a progressive accumulation of changes that are ultimately responsible for “ever-increasing susceptibility to disease and death” (Harman, 1981). His “free-radicals theory of aging” (1956) posited that aging, as well as age-associated degenerative diseases, is a consequence of free radicals (the ROS produced by mitochondria) attacking cells and tissues. Specifically, an imbalance between ROS production and antioxidant defenses (another mitochondria function) can impair cellular function. This phenomenon has been observed not only in normal aging but in many pathological cases involving mitochondrial dysfunction (see Grimm & Eckert, 2017).
In addition, cells lose their ability to recycle organelles and macromolecules with advancing age. This too leads to mitochondrial dysfunction, evidenced by impaired ability to provide energy to cells and increased oxidative stress that, in turn, damages mitochondria Rango & Bresolin, 2018. Mutations in our mitochondrial DNA also increase as we age and can eventually lead to cells’ death (see Rango & Bresolin, 2018).
Other researchers have associated aging with mutations in mitochondrial DNA in the dopaminergic neurons of a specific region of the brain, the substantia nigra (e.g., Kraytsberg et al., 2006). Similar mutations are found in patients with Parkinson’s disease (Rango & Bresolin, 2018), a neurodegenerative disorder with symptoms such as rigidity and resting tremor. In primates, neuronal senescence (a process by which neurons age and stop dividing but do not die) has been associated with altered mitochondrial function and motor decline: Older animals had less motor activity than younger animals and reduced ATP synthesis in the substantia nigra and putamen (Pandya et al., 2015). These findings are especially relevant because about half of individuals over 85 years old show mild signs of Parkinson’s (Biskup & Moore, 2006). Overall, changes associated with mitochondria dysfunction appear to be linked to both Parkinson’s and aging (Rango & Bresolin, 2018).
Further, damaged mitochondrial DNA in the substantia nigra may contribute to some movement issues that arise with age, along with other aging outcomes. In a study with mice, researchers found evidence that rodents with damaged mitochondrial DNA showed decreased dopaminergic neurons and signs of aging that included osteoporosis, kyphosis (i.e., hunchback), and weight loss (Trifunovic et al., 2004).
Research has also shown that mitochondria change their shape through regulated processes of fusion and fission (lengthening and shortening, respectively) and actively move between neuron parts (e.g., Chan, 2012). These morphological and functional changes are affected by extracellular signals and the metabolic environment and may influence the cellular aging process.
In a 2014 article in Proceedings of the National Academy of Sciences, Yuko Hara (Icahn School of Medicine at Mount Sinai) and colleagues explored the relationship between aging, mitochondria morphology, and cognition in rhesus monkeys. They found that in the brain area associated with working memory, young monkeys had normally shaped mitochondria, whereas older monkeys had many abnormally shaped mitochondria as a result of oxidative stress. The presence of these abnormally shaped mitochondria was associated with declines in working memory. However, estradiol, a hormone with an antioxidant effect, was shown to revise both this presence and working memory impairment. Thus, Hara and colleagues’ findings suggest that “hormone replacement therapy benefits cognitive aging, in part, by promoting mitochondrial and synaptic health in the PFC [prefrontal cortex].”
Neurodegenerative diseases and mitochondrial dysfunction
Age is the main risk factor for neurodegenerative disorders (Niccoli & Partridge, 2012), including Alzheimer’s disease, which starts with cognitive symptoms such as difficulty remembering recent events and, as the disease progresses, results in loss of bodily functions and death. The prevalence of Alzheimer’s among Americans 65 or older is expected to grow from 6 million today to 12.7 million by 2050, barring medical breakthroughs to prevent, slow, or cure the disease (see the Alzheimer’s Association’s 2021 Alzheimer’s Disease Facts and Figures report). Unsurprisingly, mitochondria have also been implicated in Alzheimer’s disease.
One of the earliest signs of Alzheimer’s disease, appearing even before the onset of histopathological markers and symptoms, is a reduced uptake of glucose, consistent with changes in the brain’s bioenergetic processes (Gibson & Shi, 2010). Studies have indicated that in patients with Alzheimer’s disease, the rate of glucose metabolism in brain regions involved in memory processes, such as the hippocampus and temporal and parietal lobes, is reduced by 20% to 30% (Kapogiannis & Mattson, 2011). Although research is ongoing, some investigators have proposed the “mitochondrial cascade hypothesis” to explain the onset of Alzheimer’s (Swerdlow & Khan, 2004). According to this hypothesis, genetic factors influence a host of mitochondrial functions that, in turn, convey susceptibility to Alzheimer’s. Consequently, mitochondrial dysfunction is the primary trigger of the cascade of events that lead to the disease. This hypothesis is still being tested, but mitochondrial morphology and number, bioenergetic processes, mitochondrial biogenesis (i.e., the formation of new mitochondria), and mitochondrial transport and destruction (i.e., mitophagy) do all appear to be impaired in patients with Alzheimer’s disease (for a review, see Cenini & Voos, 2019).
Keeping mitochondria healthy
Although research on mitochondria and their relationship with aging is still nascent and cannot offer conclusive recommendations, two interventions have shown promise for improving the outcomes associated with aging mitochondria: targeted pharmacological approaches, such as antioxidants (e.g., ginkgo biloba), and lifestyle changes (Cenini & Voos, 2019).
Regarding lifestyle changes, Martin Picard (University of Pennsylvania) and APS William James Fellow Bruce S. McEwen (The Rockefeller University) suggested in a 2014 commentary in Proceedings of the National Academy of Sciences that exercise and other forms of physical activity may benefit the brain by supporting healthy mitochondrial dynamics and inducing mitochondrial biogenesis. “Our repertoire of approaches to promote brain adaptability and preserve brain function with aging should benefit from a bioenergetic perspective of how the brain works and dynamically responds to its environment,” Picard and McEwen wrote.
Likewise, Kramer and Bressan highlighted the importance of lifestyle choices for slowing down aging-related declines.
Sleep may help to detoxify the brain by eliminating beta-amyloid, a substance that harms neurons and mitochondria when accumulated in excess (Huang et al., 2012). Lack of sleep can damage mitochondria, interfere with learning and memory, and ultimately lead to dementia and death (see Kramer & Bressan, 2018).
Exercise appears to keep mitochondrial DNA healthy, with fewer mutations, among other benefits (Cao et al., 2012). During prolonged exercise, the body and brain continue to expend energy while the usual source of that energy, glucose, is depleted. When this happens, mitochondria must use a different “fuel,” creating a chain of reactions that ultimately trigger the production of brain-derived neurotrophic factor (BDNF), a protein that stimulates the growth and repair of neurons and synapses.
Eating moderately may also promote mitochondrial reproduction and ATP generation and protect cells, including neurons. These effects probably occur via the same glucose-depletion mechanism that operates when one exercises (e.g., Gano et al., 2014).
A balanced diet can also reduce mitochondria’s oxidative stress and contribute to healthier mitochondria and cells (Cenini & Voos, 2019). For instance, the Mediterranean diet—characteristically high in olive oil, unrefined grains, fruits, vegetables, and fish—appears to be associated with a reduced incidence of Alzheimer’s disease (e.g., Karstens et al., 2019).
Stress management (e.g., through meditation or other relaxation techniques) can reduce free-radical damage and protect mitochondria, supporting the production of ATP and its use by cells (Bhasin et al., 2013). Chronic and intense stress can counteract BDNF, increase free-radical production, and reduce mitochondria’s capacity to create ATP and lock in the calcium needed for cellular energy production.
Moving beyond these approaches, a better understanding of the mitochondria in the context of aging, particularly brain aging, may help to identify therapeutics that could improve aging outcomes and even prevent neurodegeneration (Grimm & Eckert, 2017). Such understanding may be possible only if interdisciplinary teams including biologists, neuroscientists, and psychological scientists, among others, work together to learn more about the central role that mitochondria appear to play in all human functioning. Placing mitochondrial functioning at the core of many physical, psychiatric, and neurological diseases, as well as normal aging, “may be useful for identifying genetic and environmental influences on the development of intelligence and rate of age-related declines in cognition,” wrote Geary in his 2019 article in Current Directions in Psychological Science. What’s more, he continued, “the emerging mitochondrial therapies for specific diseases (e.g., Alzheimer’s) might prove to be broadly useful, specifically, for the amelioration of environmental and age-related compromises to health and cognition.”
References
Alzheimer’s Association. (2021). 2021 Alzheimer’s disease facts and figures. Alzheimer’s & Dementia, 17(3), 327–406. https://doi.org/10.1002/alz.12328
Bélanger, M., Allaman, I., & Magistretti, P. J. (2011). Brain energy metabolism: focus on astrocyte-neuron metabolic cooperation. Cell Metabolism, 14(6), 724–738.
Biskup, S., & Moore, D. J. (2006). Detrimental deletions: Mitochondria, aging and Parkinson’s disease. Bioessays, 28(10), 963–967. https://doi.org/10.1002/bies.20471
Boesch, P., Weber-Lotfi, F., Ibrahim, N., Tarasenko, V., Cosset, A., Paulus, F., Lightowlers, R. N., & Dietrich, A. (2011). DNA repair in organelles: Pathways, organization, regulation, relevance in disease and aging. Biochimica et Biophysica Acta—Molecular Cell Research, 1813(1), 186–200. https://doi.org/10.1016/j.bbamcr.2010.10.002
Cao, X., Zhao, Z. W., Zhou, H. Y., Chen, G. Q., & Yang, H. J. (2012). Effects of exercise intensity on copy number and mutations of mitochondrial DNA in gastrocnemus muscles in mice. Molecular Medicine Reports, 6, 426–428. https://doi.org/10.3892/mmr.2012.913
Cenini, G., & Voos, W. (2019). Mitochondria as potential targets in Alzheimer disease therapy: An update. Frontiers in Pharmacology, 10, Article 902. https://doi.org/10.3389/fphar.2019.00902
Chan, D. C. (2012). Fusion and fission: Interlinked processes critical for mitochondrial health. Annual Review of Genetics, 46, 265–287. https://doi.org/10.1146/annurev-genet-110410-132529
Deheshi, S., Pasqualotto, B. A., & Rintoul, G. L. (2013). Mitochondrial trafficking in neuropsychiatric diseases. Neurobiology of Disease, 51, 66–71. https://doi.org/10.1016/j.nbd.2012.06.015
Ernster, L., & Schatz, G. (1981). Mitochondria: A historical review. Journal of Cell Biology, 91(3), 227s–255s.
Frye, R. E., & Rossignol, D. A. (2011). Mitochondrial dysfunction can connect the diverse medical symptoms associated with autism spectrum disorders. Pediatric Research, 69(8), 41–47. https://doi.org/10.1111/j.1469-8749.2011.03980.x
Gardner, A., & Boles, R. G. (2011). Beyond the serotonin hypothesis: Mitochondria, inflammation and neurodegeneration in major depression and affective spectrum disorders. Progress in Neuro-Psychopharmacology and Biological Psychiatry, 35(3), 730–743. https://doi.org/10.1016/j.pnpbp.2010.07.030
Geary, D. C. (2019). The spark of life and the unification of intelligence, health, and aging. Current Directions in Psychological Science, 28(3), 223–228. https://doi.org/10.1177/0963721412469398
Green, D. R., & Reed, J. C. (1998). Mitochondria and apoptosis. Science, 1309–1312. https://doi.org/ 10.1126/science.281.5381.1309
Grimm, A., & Eckert, A. (2017). Brain aging and neurodegeneration: From a mitochondrial point of view. Journal of Neurochemistry, 143(4), 418–431. https://doi.org/10.1111/jnc.14037
Harman, D. (1981). The aging process. Proceedings of the National Academy of Sciences, 78(11), 7124–7128.
Huang, Y., Potter, R., Sigurdson, W., Santacruz, A., Shih, S., Ju, Y.-E., Kasten, T., Morris, J. C., Mintun, M., Duntley, S., Bateman, R. J. (2012). Effects of age and amyloid deposition on Aß dynamics in the human central nervous system. Archives of Neurology, 69, 51–58. https://doi.org/10.1001/archneurol.2011.235
Kaplan, B. J., Rucklidge, J. J., Romijn, A., & McLeod, K. (2015). The emerging field of nutritional mental health: Inflammation, the microbiome, oxidative stress, and mitochondrial function. Clinical Psychological Science, 3(6), 964–980. https://doi.org/10.1177/2167702614555413
Karstens, A. J., Tussing-Humphreys, L., Zhan, L., Rajendran, N., Cohen, J., Dion, C., Xiahong, J. Z., & Lamar, M. (2019). Associations of the Mediterranean diet with cognitive and neuroimaging phenotypes of dementia in healthy older adults. American Journal of Clinical Nutrition, 109(2), 361–368. https://doi.org/10.1093/ajcn/nqy275
Kramer, P., & Bressan, P. (2018). Our (mother’s) mitochondria and our mind. Perspectives on Psychological Science, 13(1), 88–100. https://doi.org/10.1177/1745691617718356
Kraytsberg, Y., Kudryavtseva, E., McKee, A. C., Geula, C., Kowall, N. W., & Khrapko, K. (2006). Mitochondrial DNA deletions are abundant and cause functional impairment in aged human substantia nigra neurons. Nature Genetics, 38(5), 518–520.
Niccoli, T., & Partridge, L. (2012). Ageing as a risk factor for disease. Current Biology, 22(17), R741–R752. https://doi.org/10.1016/j.cub.2012.07.024
Pagliarini, D. J., & Rutter, J. (2013). Hallmarks of a new era in mitochondrial biochemistry. Genes & Development, 27(24), 2615–2627. https://doi.org/10.1101/gad.229724.113
Pandya, J. D., Grondin, R., Yonutas, H. M., Haghnazar, H., Gash, D. M., Zhang, Z., & Sullivan, P. G. (2015). Decreased mitochondrial bioenergetics and calcium buffering capacity in the basal ganglia correlates with motor deficits in a nonhuman primate model of aging. Neurobiology of Aging, 36(5), 1903–1913. https://doi.org/10.1016/j.neurobiolaging.2015.01.018
Picard, M., & McEwen, B. S. (2014). Mitochondria impact brain function and cognition. Proceedings of the National Academy of Sciences, 111(1), 7-8. https://doi.org/10.1073/pnas.1321881111
Rango, M., & Bresolin, N. (2018). Brain mitochondria, aging, and Parkinson’s disease. Genes, 9(5), 250. https://doi.org/10.3390/genes9050250
Swerdlow, R. H., & Khan, S. M. (2004). A “mitochondrial cascade hypothesis” for sporadic Alzheimer’s disease. Medical Hypotheses, 63(1), 8–20. https://doi.org/10.1016/j.mehy.2003.12.045
Youle, R. J., & van der Bliek, A. M. (2012). Mitochondrial fission, fusion, and stress. Science, 337(6098), 1062–1065. https://doi.org/10.1126/science.1219855
APS regularly opens certain online articles for discussion on our website. Effective February 2021, you must be a logged-in APS member to post comments. By posting a comment, you agree to our Community Guidelines and the display of your profile information, including your name and affiliation. Any opinions, findings, conclusions, or recommendations present in article comments are those of the writers and do not necessarily reflect the views of APS or the article’s author. For more information, please see our Community Guidelines.
Please login with your APS account to comment.